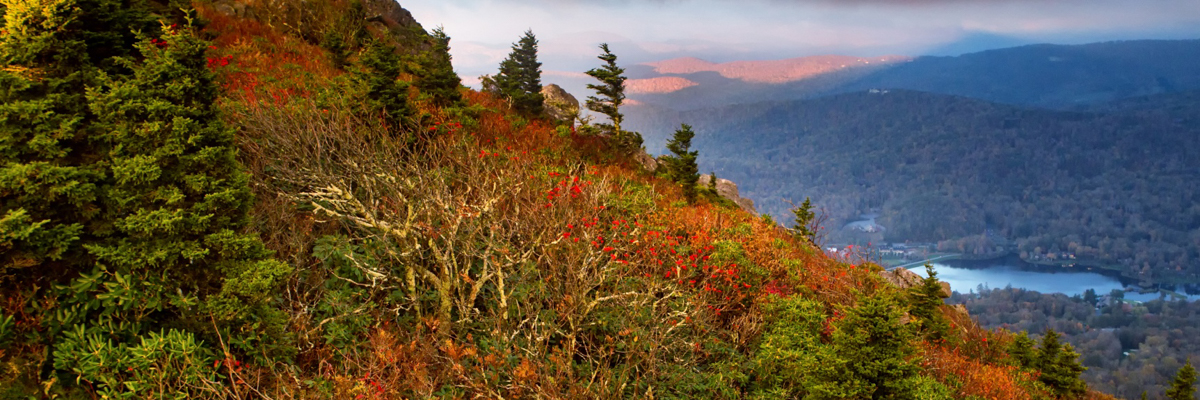
It's sometimes hard to remember that the beauty we see in fall colors is the result of the death of a tree's leaves. That there can be such joy in celebrating the death of something is a curious aspect of life worthy of a philosopher's attention. But even without the philosophical implications, we can learn much about life by studying death. In fact, when you think about in the larger context, every essay about fall colors is about death in some form. Today's essay is no exception. It focuses on the simple question of why some turn yellow and orange before they fall off and die.
Some Tree Species Always Have Yellow to Orange Leaves in the Fall
Each fall, the leaves of sugar and striped maples, hickories, beeches, birches, tulip poplars all turn a brilliant yellow color. For many sugar maples, that yellow soon gives way to a fiery orange, especially in those leaves that receive the most sunlight. The pictures below were taken by myself on October 23rd of this year of a sugar maple in Boone and you can see that the shaded leaves were yellow, while those exposed to full sun were orange-red.
Why do these trees show these particular fall colors? What pigments provide those colors, and why do we only see them in the fall? These and the answers to other questions are addressed below.
Yellow and Orange Colors are Revealed When the Chlorophyll Degrades
As I have noted in several previous essays, the yellow and orange colors in leaves are revealed when chlorophyll, the pigment responsible for making leaves appear green, is lost from the leaf. During the summer, these pigments were masked by the chlorophyll. When a tree produces a deep orange-red color, it might also be synthesizing anthocyanins, which are a different class of compound and which provide the classic red to purple color in fall leaves. However, in this essay, we will concentrate solely on the yellow and orange pigments. An earlier essay of mine discussed the synthesis and function of anthocyanins (see the archive section of my fall color page: http://biology.appstate.edu/fall-colors).
Yellow and Orange Pigments are Carotenoids
The yellow and orange pigments in fall leaves are known as carotenoids. Plants contain a variety of such compounds, but the most common are β-carotene (pronounced beta-carotene, the same pigment responsible for coloring carrots orange) and lutein (a yellow compound at low concentrations, but orange-red when concentrated) as well as some others described below. Figure 1 shows the structures of these two pigments.
Figure 1. Top structure is β-carotene and bottom structure is lutein.
A particularly good website on carotenoid chemistry can be found at: http://www.chm.bris.ac.uk/motm/carotenoids/carotenoids.htm. Regarding the structural diagrams above, it will help to know that each line represents a chemical bond whereby one electron is shared by two atoms. If there are two lines, then two electrons are shared, and we call that a double bond. Double bonds are usually stronger than single ones and do not rotate. That is, if there are atoms on one side of a double bond, they stay on that side. Contrast that with a single bond, which may rotate. In this case, an atom that is one moment on top may in the next be on the bottom.
How Carbon Bonds to Other Atoms
Where ever lines meet, there is a carbon (C) atom, even though it is not explicitly shown. This is the chemist's shorthand for drawing chemical structures. When an atom is not carbon, then its acronym is used (e.g., N for nitrogen, O for oxygen, H for hydrogen, and so on). A single C atom has four bonds it may share with other atoms. If a C atom has two double bonds, that fills up all four of its available spots for electrons and no more atoms may be joined to that carbon. If all the bonds on a carbon are single bonds, then up to four different atoms may be connected to it. For example, one carbon atom bonded to four hydrogens (CH4) is methane, one of the important greenhouse gases that contributes to global warming. When carbon shares two double bonds with oxygen, we have carbon dioxide (CO2). This versatility, along with the bond strength, may be why carbon serves as the central atom on which to base all life.
There are Two Main Types of Carotenoids
Typical carotenoids, such as β-carotene, contain only C atoms and H atoms (see Figure 1 above). However, another class of carotenoids, known as xanthophylls, also contains O atoms in addition to the C and H. This gives them additional properties especially with regards to accepting or donating electrons and the ability to dissipate energy as heat (more on that later in this essay). Lutein is a typical xanthophyll.
Figure 2. Chloroplast ultrastructure. Courtesy of Wikipedia.com.
Carotenoids are Found in Chloroplasts
Carotenoids are fat soluble pigments, meaning they do not readily dissolve readily in water. Instead, they are usually found attached to proteins or membranes in the chloroplasts. Chloroplasts (see Figure 2) are tiny organelles in cells where photosynthesis occurs. Because they contain a special primitive form of DNA, and have a double outer membrane (the outer one would be from the host cell, while the inner one is structurally similar to that of a bacterial cell membrane), we think they were derived from photosynthetic bacteria billions of years ago. They are also approximately the same size as a bacterium. Carotenoids are an ancient class of pigments, thought to have evolved perhaps 3 billion years ago.
Figure 3. Spectral absorbance curve for chlorophyll and carotenoids. Image courtesy of D.W. Reed, TAMU.
Carotenoids Have Two Primary Functions in Leaves
One function of carotenoids is to absorb light in wavelengths that chlorophyll is inefficient at absorbing, such as the blue-green to green wavelengths. Figure 3 shows the absorption spectrum for chlorophyll and carotenoids. Along the horizontal line is the range of wavelengths that correspond to visible light, and which is nearly the same as that used by plants for photosynthesis. Notice that short wavelengths correspond to the blue portion of the spectrum, while longer wavelengths encompass the red portion. You can easily see that chlorophyll preferentially absorbs the blue and red wavelengths, and does poorly in the green range. That is why leaves appear green, because light reflected from leaf to your eye is enriched in the green wavelengths relative to the blue or red.
Figure 4. Chlorophyll antennae and associated pigments molecules (carotenoids). Image courtesy of http://www.bio.umass.edu/biology/conn.river/light.html.
Carotenoids Absorb In Wavelengths that Chlorophyll Does Poorly In
Meanwhile, the carotenoids are absorbing maximally at those wavelengths where chlorophyll does poorly (light blue to green). Once that light energy is absorbed, the carotenoids pass that energy on to a neighboring chlorophyll molecule. In the leaf, chlorophyll molecules and carotenoids are situated near each other in clusters, somewhat analogous to a dish antenna (see Figure 4). This physical arrangement maximizes the capture of a photon (a packet of light energy, a concept courtesy of quantum physics), because if chlorophyll molecules were just individually arraigned throughout the chloroplast, most photons would miss them and the potential to harness their energy would be wasted.
Carotenoids are More than Just Accessory Pigments
Because carotenoids assist in absorbing photons for photosynthesis, they have been called accessory pigments. But over the past 30 years, it has become apparent that they have a second function, no less important than the first. And that is to divert excess energy away from the chlorophyll molecules. This is exactly opposite of its functioning as I have just described it, which may seem confusing. But in fact, it makes great sense. When too much light strikes a leaf, that energy has to be dissipated. When just enough light reaches a leaf, it is used to move electrons and protons so the leaf can make sugars during photosynthesis.
But if too much energy comes in, the electron transport chain (ETC), which is responsible for moving the electrons, gets overloaded. A note here: the ETC is not really a chain in the literal sense. Rather, it is a series of molecules that alternately accept or donate electrons, all the while moving them in a single direction. Think of it as a bucket brigade at a fire, where each person transfers a bucket of water to the next person, except that instead of moving water, the molecules move electrons. When the ETC gets overloaded, bad things can happen. In one case, electrons that are energized when light strikes the chlorophyll molecule can be dumped onto oxygen molecules, creating a type of new type of oxygen known as superoxide (O2-, essentially oxygen with an extra negative charge). This is a very dangerous molecule, because it can react with membranes and proteins in the chloroplast, causing severe damage and eventual death. In another case, an excited chlorophyll may cause the formation of singlet oxygen, which is also highly reactive (but not charged). This molecule too can wreak havoc within the leaf. When this happens, anti-oxidants are brought in which render these compounds (called reactive oxygen species, or ROS) inert.
Carotenoids can Dissipate Excess Energy as Heat
In leaves, carotenoids can accept the energy from an excited chlorophyll molecule and dissipate that energy as heat. This happens because the carotenoid is especially good at vibrating when it absorbs this excess energy, and that results in the loss of heat (just like if you rapidly bend a piece of metal—it gets hot and the energy of bending is given off as heat). If the excess light energy is released as heat, it is no longer available to make those nasty ROS, and the leaf is protected from photodamage.
Plants Can Regulate the Degree to Which Carotenoids Dissipate Excess Energy as Heat
What is particularly interesting is that leaves can regulate their potential to dissipate excess energy depending on the environmental conditions. Consider a leaf growing in deep shade. At intervals throughout the day, direct light may reach that leaf by finding a way through a hole in the canopy above. We call this a sunfleck. Although most sunflecks are of short duration, some can last for minutes to hours, and can greatly stress a shade leaf, especially one that is physiologically adapted to low light. When this bright light hits the leaf, it causes an imbalance between energy capture (the conversion of light energy into chemical energy) and energy utilization (the making of sugars in photosynthesis). In turn, this causes certain spaces inside a chloroplast to become acidic (which means that protons accumulate). Protons are simply hydrogen atoms missing their one electron. The more protons, the more acidic is a solution, and the lower the pH.
Figure 5. The xanthophyll cycle. As you go from zeaxanthin to violaxanthin, notice how the ring structures each gain an oxygen. This is known as de-epoxidation. Violaxanthin can dissipate excess light energy as heat by vibrating rapidly, whereas zeaxanthin cannot. pH appears to control this conversion—when photosynthetic electron transport is high, it tends to lower the pH. A low pH acts as a signal to start de-epoxidation. Antheraxanthin is an intermediate compound in this cycle. When stress conditions are relieved, pH rises, and the violaxanthin is converted back to zeaxanthin, in a process known as epoxidation.
Acid Conditions Signal the Leaf to Activate the Xanthophyll Cycle
Acidic conditions stimulate the production of enzymes that convert a special xanthophyll known as zeaxanthin (which is yellow) into a new compound known as violaxanthin (which is orange) through the intermediate compound antheraxanthin. Figure 5 shows this conversion scheme. Note that the conversion involves adding an oxygen molecule to each of the two six-sided rings on either side of the zeaxanthin, a process known as de-epoxidation.
Violaxanthin is the compound that dissipates the excess light energy as heat. As long as the leaf is stressed, this compound is retained in the chloroplast, and energy is diverted away from chlorophyll to be lost as heat. When the sunfleck passes, and the leaf returns to low light conditions, the acidity that built up decays away, the cycle reverses, and the violaxanthin converts back to zeaxanthin via an epoxidation reaction. This way, the leaf does not divert energy away from photosynthesis when light is limiting, but does when it is in excess. Thus, this system acts like a pressure release valve, except that instead of steam being released, it is the energy of the photons.
Carotenoids May Also Protect the Leaf in the Fall
As chlorophyll degrades in the fall, light energy impinging on the leaf can cause injury to the internal biochemical machinery, especially the parts responsible for withdrawing nutrients back into the leaf. The presence of the carotenoids may help the leaf dissipate this excess energy via the xanthophyll cycle, or, they may physically shield the proteins and membranes by acting as a light screen, which may assist the leaf in withdrawing nutrients back into the twigs so that the tree can reuse them next season when it forms new leaves.
Fall Colors May Act as a Signal of a Healthy Ecosystem
So as we have seen, the beauty of fall color is not just an arbitrary act for our visual pleasure. Rather, the presence of these pigments shows that they are working to protect the leaf. If the leaves are protected as they die, that ultimately affects the health and vigor of the tree. Healthy trees, in turn, are the basis for maintaining healthy ecosystems. So, fall color may be a not-so-subtle signal of the health of our forests. And that is something worth knowing!