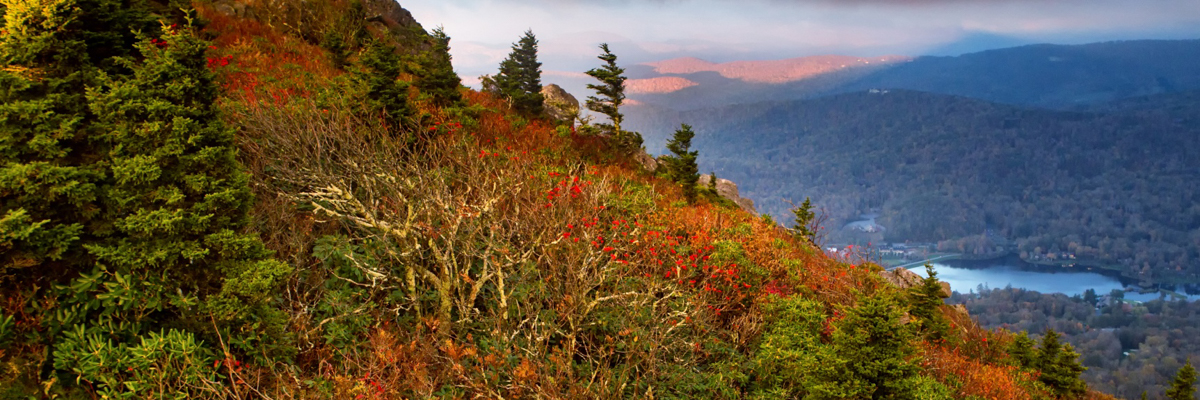
Fall Color Report for August 31, 2014
For those who want a short (< 500 words) summary of the state of fall leaf colors here in the mountains of western NC, I will post my observations at the beginning of my report each week. Then, below the report, I will provide an essay on trees that some of you may find interesting. This way, you can get right to the fall color report and not have to wade through my science essays. This past week, the weather has moved back to a more normal pattern for this time of year and we're back to our usual "dog days" of August. As a result, there is not much to report with respect to fall colors aside from what I wrote last week. The forests here are still very green. Jesse Pope reports from Grandfather Mountain that: "We do have some more leaf change on red maples, and also just plain leaf loss on them. Many of the maple trees above 4800 ft have already shed 50% of their foliage. Not sure what's going on there. No other trees are changing drastically yet. The buckeyes are starting to show just a smidge of color but nothing too drastic. I think we are still a couple weeks out from any real noticeable changes here at Grandfather." Kathy Mathews, from Western Carolina University, and Jonathan Horton, from UNC-Asheville, also report little progress to date on fall colors around Asheville and Cullowhee. Jonathan did mention yellowing on tulip poplars in the Bent Creek Experimental Forest (a unit of the US Forest Service that has some nice walking and biking trails, and which is adjacent to the NC Arboretum), and he saw some few Virginia creepers turning red. I think it must be drier at lower elevations, as I also saw many tulip poplars in the Piedmont this weekend with yellow leaves. So, leaf lookers, hang in there. Over the next two weeks, we should begin to see trees turning at the highest elevations (5,000 ft or so) and then watch it progress downwards each week.
To See Pictures, visit the Fall Color Guy's Facebook page!
Tree Science – How Trees Use Water
Last week I gave a fairly extended explanation of how water moves from the roots of a tree to the leaves and out into the atmosphere. This week, I'd like to explain what physical and biological factors affect the rate at which that water moves through the trees. This will involve some knowledge of fluid dynamics and plant physiology, but hang in there; it's actually fun to know!
My mantra here is that structure and function are tightly intertwined. That means we have to learn the structure of the cells that transport the water if we want to understand why they function like they do. The cells that conduct water in plants are known as xylem cells and we're all familiar with them –tree xylem, for example, is wood! Interestingly, xylem cells are dead at maturity, and this contributes to their efficiency. Being dead, they have no cellular contents and are essentially just hollow structures with walls made out of cellulose and lignin, the two main components of wood. The walls provide rigidity to the cells and contribute to the ability of trees to grow to great heights without crushing themselves. The lack of cell contents allows these cells to conduct water with high efficiency.
Conifers, which evolved prior to the emergence of angiosperms (the flowering plants), contain a primitive type of xylem cell called a tracheid. Tracheids are long, narrow, and hollow cells, ranging 10-30 um in diameter). Figure 1 shows a typical tracheid. They also have end walls. For water to move from cell to cell, it has to pass through small openings in the wall, called pit pores (see Figure 2). As we will see below, the presence of end walls and having to move through the pits present obstacles to the movement of water for these types of plants.
Angiosperms, on the other hand, in addition to tracheids, contain a more recently evolved xylem cell called a vessel element (see Figure 1 again). These cells are much wider (100 up to 800 um diameter), shorter, and often have no cell walls on either end. Vessel elements also contain pits and water can not only move through the vessel element, but can cross over to other vessel elements through the pits.
Vessel elements can be stacked together to form what we call "vessels". An analogy would be if you stacked toilet paper tubes one on top of the other. Each tube would be a vessel element and the set of joined tubes a vessel. A vessel is bounded on either end by vessel elements that do have a porous end wall. Such walls may act to restrict the movement of air bubbles in the xylem. Some vessels are just two or three cells long, whereas in some tree species, such as ash trees, they can be 10 m long! The evolution of vessel elements probably contributed to the ability of flowering plants to dominant certain landscapes, as we'll discuss below.
At this point, we know something about the anatomy of these xylem cells, but how does their anatomy affect the movement of water through them? For that, we turn to the engineering field of fluid dynamics. Luckily, we can handle this type of engineering verbally and without formal mathematical equations (although I'll show an equation to you anyway, for those of you who like math).
If you've ever been to a fast food restaurant, you've probably seen those small stir straws. Few of you would want to use those straws in your drink, because you know that it would be hard to suck the drink up the straw. In contrast, if you had a regular straw, with its much larger diameter, it would be much easier to get the drink into your mouth. Why is that?
Well, we need to know two things about how water flows in tubes before we can figure this out. First, water along the sides of the straw will rub against the walls, creating friction, and that will slow these water molecules down compared to those in the center of the straw. Second, water in the middle of the straw is drawn up the straw by the vacuum applied when you suck on the straw, and the entire column of water moves because of cohesion with other water molecules.
Now, that single layer of water at the straw's edge that is moving slower will slow down the next layer inward of water because of shear, which is what happens when one layer of water rubs against another. That layer will interact with the next most layer, and slightly slow it down too. However, because water is somewhat slippery the frictional resistance of one layer of water against another is much smaller than that with the walls of the straw and as one moves to the center of the straw, these effects are drastically reduced. As a result, water moves faster at the center of a tube than at the edges. See Figure 3, which shows the velocity of water as a function of the distance from the side of a tube.
If you have a very narrow tube, a greater percentage of all the water in the tube will be interacting with the sides and as a result, more of the water in the straw will be moving slowly. Going back to the straw analogy, it means you will have to suck much harder to get the same flow rate in a narrow straw as compared to a wide straw, since you have to overcome more frictional resistance. Therefore, to get the same flow rate in a narrow tube as in a large one, you have to exert a greater tension (or pressure if you're pushing the water). In fact, from a fluid dynamics point of view, if you keep the pressure constant, but decrease the radius of a tube by half, you reduce the flow by 16 times! Conversely, if you double the radius, flow increases by 16 times! Mathematically, we're saying that the flow of water is proportional the radius raised to the fourth power: F α r4. This means that doubling the radius is the same as raising two to the fourth power (24 = 16).
For a tree, this has enormous consequences. It means that at any given tension in the xylem, it will be the larger diameter xylem cells that are conducting most of the water. If you compare tracheids to vessel elements, the implications are obvious: flowering plants (the only ones to have evolved vessel elements) will have a much greater capacity to move water (at equivalent tensions) than will conifers and ferns, which have only the much narrower tracheids. In fact, the fastest sap in the world has been found in the kiwi vine, which has vessel elements nearly 800 um wide (or 0.8 mm). This down-under vine can have rates of flow exceeding 20 mm/s (>50 m/h or 164 ft/h). In fact, kiwi vines have the fastest sap in the west (and north, and south, and east!). In deciduous trees, whose xylem diameters are usually less than 500 mm, and often between 100 and 400 um, rates of flow are slightly lower (25 to 40 m/h or 82 to 131 ft/h).
In contrast, conifers and ferns, with their more primitive and narrower tracheids, cannot move water nearly as fast. Rates of movement in pine trees, for example, are only around 1 mm/s (~6 m/h or ~20 ft/h). Why are sap velocities so much higher in vines and deciduous trees? Well, remember, vessel elements are much wider than tracheids, and a much smaller percentage of the water is in contact with the walls. This means the water can flow more freely, and less tension is needed to move large volumes up the plant. Also, the lack of end walls further reduces the tortuosity of the pathway (how contorted a path the liquid must traverse), and instead of having to move primarily through pits, as water must do in tracheids, it can flow freely down the wide, hollow vessels.
Mathematical Diversion for the Adventurist: You can confirm mathematically why water flows faster through vessels than tracheids if you remember your geometry. For a cell with a radius of r, the circumference (which you can consider the single layer of water touching the walls), will be: C = 2πr. For a tracheid that has an r=10 um, C = 63 um. The volume of water in a cell can be approximated by the cross-sectional area (A) of the cell, and the formula for that is A=πr2. For this cell, A=314 um2. If you have a vessel element with r=125 um, C = 785 um and A=49,807 um2. Now, take the ratio of A to C for both cells to get an idea of how much water is in the cell compared to how much is interacting with the walls. For the tracheid, the ratio is 314/63 = ~5. For the vessel element, it is 49,807/785 = ~63. Thus you can see that there is about 12 times more "free" water in a vessel element than in a tracheid, which is why water can flow faster in a wider tube (i.e., cell!).
The large frictional resistance in tracheids greatly slows down the velocity of the sap in ferns and conifers. This means that in response to a large transpirational demand (i.e., a lot of dry air out there), angiosperms will be able to transport water more easily and faster to the leaves than will conifers. Also, when water is plentiful, angiosperms will have the luxury of being able to open their stomata to take in carbon dioxide for photosynthesis, and not have to "worry" about all the water that is being lost via transpiration in that process. If a conifer did this, the loss of water from the needles would exceed the capacity of the xylem to replace the water lost via transpiration, and the tree would dry out. Of course, this is a greatly simplified explanation, but you get the general idea of how wood anatomy can determine how trees move water in their trunks.
Figures from: http://plantsstructureandreproduction77.wikispaces.com/structure2 and pits in the walls of a softwood tracheid (hollow fibre). in Keey et al. 2000.
Next week: What happens to a tree if it gets drought stressed? Is there a trade-off between xylem efficiency and safety? How does evolution solve such a dilemma?